04/07/20
Evelyn Lamb

The ADMX experiment trains scientists to deal with real signals—by creating fake ones.
The Axion Dark Matter Experiment searches for dark matter the way you might search for a radio station in an unfamiliar location. In a process that takes quite a bit longer than simply turning the dial, it scans across frequency bands that correspond to the possible masses of the particle they’re looking for. If they get a hint on their first pass—metaphorically, a few notes that sound like the kind of music they’d like to hear—they conduct a more thorough analysis of that frequency.
They usually do get a few hints on each pass, says University of Washington physicist Gray Rybka, co-spokesperson of ADMX. Some of this is due to random signal fluctuation. Some of it is due to leaky radio signals. (At one point it was a local religious broadcaster. “We received a message from God,” Rybka jokes.)
And some of it is actually a test: A small subset of ADMX scientists are responsible for injecting synthetic signals into the data.
A tricky signal
Dark matter, so called because it does not interact with light or other electromagnetic radiation, explains many observations about the distribution and movements of stars and galaxies. Astrophysicists estimate that it makes up 85% of the total matter of the universe, but they don’t know what it is. “Everything in our zoo of particle physics—every particle we know of—does not fit the bill,” Rybka says
The axion is one of several dark matter candidates. The particle was originally proposed in the 1970s as a potential solution to the strong CP problem in particle physics. Later, researchers saw that the particle could also explain dark matter.
“This is two for one,” says ADMX analysis team member Leanne Duffy of the US Department of Energy’s Los Alamos National Laboratory. “Not only do you solve this existing problem with the Standard Model, but you also get an excellent dark matter candidate out of it.”
Assuming dark matter axions exist, the Earth and everyone on it is traveling through a “galactic halo” that is thick with them. To touch an axion, we don’t need to do anything.
ADMX is the only one of DOE’s flagship dark matter searches looking for axions. The question is how to detect them. ADMX scientists hope to do it by converting them into particles that are much easier to detect: photons, quanta of light.
In the presence of a strong magnetic field, axions should convert into photons. ADMX creates a magnetic field and isolates waves of specific frequencies in a microwave cavity where they can record any axions-turned-photons they come across.
Passing the test
Keeping the experiment cold (less than 100 millikelvins above absolute 0) helps separate the signal from the noise by decreasing the number of background photons coming from other sources. But some still do sneak in.
To make sure the scientists are up to the task of eliminating those background signals, ADMX scientists do something that other experiments do as well—they regularly inject false signals into their data.
“There is always a part of us that is excited to see a signal because you don’t know if it’s an axion signal or an injected signal.” says Rakshya Khatiwada, a physicist at Fermilab.
When they inject synthetic signals, the team members responsible for injecting them usually reveal them after the second pass. One time in late 2018, the test proceeded further than that. Only Noah Oblath, a researcher at the Pacific Northwest National Laboratory, and one other colleague knew. “It was a little bit strange,” Oblath says. “I like generally being honest with people.”
The team proceeded with the next steps of the analysis. When the signal persisted, they had a meeting to discuss how to proceed. “Fortunately this was a teleconference, and I didn’t have any video on, so I didn’t have to worry about covering my grin or anything,” Oblath says.
They kept up the ruse this time in order to test the scientists’ reactions.
Rybka says he was doubtful. “There was nothing strange about it,” he says.
And that was the problem. The signal had been perfectly clear, and its shape was exactly what they had predicted. “When I looked at it, I said, ‘This might be too good to be true.’”
Duffy had her suspicions as well. And unlike Rybka, she had the tools to test them.
The high-resolution analysis would have exposed the injections as false immediately. But going to the high-resolution channel wasn’t part of the analysis protocol. Still, she admits, “If I hadn’t been so busy, I probably would have gone and looked at it and just not told anyone.”
On the call, the doubtful scientists couldn’t let their suspicions guide their actions. If it was a test, it was a test of their process. They began to discuss the next step: Turning the detector’s magnet off to see whether changing the magnetic field affected the signal, as they would expect if it came from a real axion.
“At that point, Gray paused and gave me a chance to reveal whether it was an injection or not,” Oblath says.
Powering the magnet down would delay the rest of the experiment, so it was time for Oblath to confess. The test had gone according to plan.
“It was a great way to test that our axion detection procedure works,” Duffy says. “But it would be nice to actually detect a real axion at some point.”
See the full article here .
Dark Matter Research
Scientists studying the cosmic microwave background hope to learn about more than just how the universe grew—it could also offer insight into dark matter, dark energy and the mass of the neutrino.




Dark Matter Background
Fritz Zwicky discovered Dark Matter in the 1930s when observing the movement of the Coma Cluster., Vera Rubin a Woman in STEM denied the Nobel, did most of the work on Dark Matter.


In modern times, it was astronomer Fritz Zwicky, in the 1930s, who made the first observations of what we now call dark matter. His 1933 observations of the Coma Cluster of galaxies seemed to indicated it has a mass 500 times more than that previously calculated by Edwin Hubble. Furthermore, this extra mass seemed to be completely invisible. Although Zwicky’s observations were initially met with much skepticism, they were later confirmed by other groups of astronomers.
Thirty years later, astronomer Vera Rubin provided a huge piece of evidence for the existence of dark matter. She discovered that the centers of galaxies rotate at the same speed as their extremities, whereas, of course, they should rotate faster. Think of a vinyl LP on a record deck: its center rotates faster than its edge. That’s what logic dictates we should see in galaxies too. But we do not. The only way to explain this is if the whole galaxy is only the center of some much larger structure, as if it is only the label on the LP so to speak, causing the galaxy to have a consistent rotation speed from center to edge.
Vera Rubin, following Zwicky, postulated that the missing structure in galaxies is dark matter. Her ideas were met with much resistance from the astronomical community, but her observations have been confirmed and are seen today as pivotal proof of the existence of dark matter.
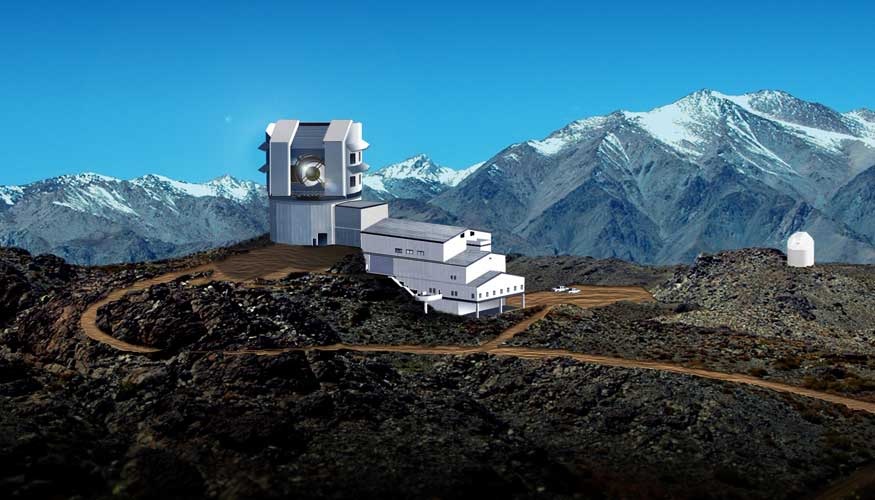

five-ways-keep-your-child-safe-school-shootings
Please help promote STEM in your local schools.